A Proof That Some Spaces Can’t Be Cut
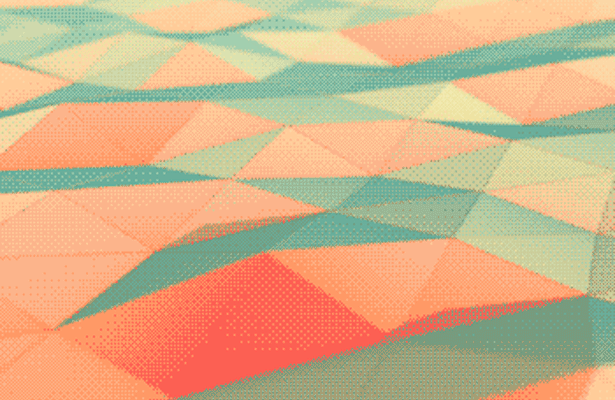
The question is deceptively simple: Given a geometric space — a sphere, perhaps, or a doughnut-like torus — is it possible to divide it into smaller pieces? In the case of the two-dimensional surface of a sphere, the answer is clearly yes. Anyone can tile a mosaic of triangles over any two-dimensional surface. Likewise, any three-dimensional space can be cut up into an arbitrary number of pyramids.
But what about spaces in higher dimensions? Mathematicians have long been interested in the general properties of abstract spaces, or manifolds, which exist in every dimension. Could every four-dimensional manifold survive being sliced into smaller units? What about a five-dimensional manifold, or one with an arbitrarily large number of dimensions?
Subdividing a space in this way, a process known as triangulation, is a basic tool that topologists can use to tease out the properties of manifolds. And the triangulation conjecture, which posits that all manifolds can be triangulated, is one of the most famous problems in topology.
Ciprian Manolescu remembers hearing about the triangulation conjecture for the first time as a graduate student at Harvard University in the early 2000s. Though Manolescu was considered a phenomenon when he entered Harvard as an undergraduate — he had distinguished himself as the only person, then or since, to notch three consecutive perfect scores in the International Mathematical Olympiad — trying to prove a century-old conjecture isn’t the sort of project that a wise student takes on for a doctoral thesis. Manolescu instead wrote a well-regarded dissertation on the separate topic of Floer homology and spent most of the first decade of his professional career giving the triangulation conjecture little thought. “It sounded like an unapproachable problem, so I didn’t pay much attention to it,” he wrote recently an email.
Others kept working on the problem, however, clawing toward a solution that remained stubbornly out of reach. Then in late 2012, Manolescu, now a professor at the University of California, Los Angeles, had an unexpected realization: The theory he’d constructed in his thesis eight years earlier was just what was needed to clear the final hurdle that had tripped up every previous attempt to answer the conjecture.
Building on this insight, Manolescu quickly proved that not all manifolds can be triangulated. In doing so, he not only elevated himself to the top of his field, but created a tool with enormous potential to answer other long-standing problems in topology.
Perfect Cuts
The 19th-century French polymath Henri Poincaré was one of the first mathematicians to think about manifolds as combinations of simple pieces glued together. For example, a two-dimensional sphere (which means just the surface of a solid ball) can be approximated by gluing together two-dimensional triangles, and a three-dimensional sphere can be approximated by gluing together three-dimensional tetrahedra. Triangles and tetrahedra are examples of more general shapes called simplices, which can be defined in any dimension.
Triangulating a manifold is useful in a number of ways. The triangulation offers a concrete way of visualizing spaces that are difficult to see. It also provides researchers with a starting point for computing an important mathematical tool called an invariant.
Mathematicians use invariants to determine whether two spaces are fundamentally equivalent. If you compute the invariants of two manifolds and get unequal results, you know the manifolds are topologically distinct. (The converse doesn’t always hold true — two distinct manifolds might share the same invariant.)
A simple way to see this is by computing an invariant called the Euler characteristic. To find the Euler characteristic of a two-dimensional surface, first divide it into any number of polygons. Now count the number of vertices, subtract the number of edges, then add the number of faces. The resulting integer will come out the same no matter how many polygons you use to triangulate the manifold. The Euler characteristic of a sphere is 2; that of a torus is 0. In two dimensions, any two manifolds with the same Euler characteristic are topologically equivalent.
Manifolds in higher dimensions also possess an Euler characteristic, but there, things don’t work out quite so neatly. In three dimensions, for instance, there are infinitely many distinct manifolds with a given Euler characteristic. Even so, triangulation remains a useful tool, which naturally gives rise to the question: Can all manifolds, in any dimension, be triangulated?
The question was first posed in the early 20th century, and the affirmative answer came to be known as the triangulation conjecture. Initially, mathematicians assumed the triangulation conjecture had to be true, and by the 1950s they’d proven that it held for all manifolds in the first, second and third dimensions. As the 20th century wore on, however, mathematicians discovered that higher-dimensional spaces lack many nice properties of lower-dimensional manifolds. This led mathematicians to suspect that the triangulation conjecture was likely false in higher dimensions, even though no one had been able to refute it with a proof.
The idea that spaces exist that can’t be divided into smaller units seems bizarrely counterintuitive. But one way to think about it is to lay triangles on a two-dimensional sphere, going around until the entire sphere is covered. Even in this simple case, it’s not immediately clear how to dovetail the final triangles with the original ones. Careful planning would be key. Add more dimensions to the scenario, and the problem of matching first simplices to last simplices becomes much more complicated.
In 1982, Michael Freedman, then at the University of California, San Diego, constructed four-dimensional manifolds that didn’t allow for a natural kind of triangulation, an accomplishment that helped propel him to a Fields Medal. A few years later, Andrew Casson of Yale University proved that these particular manifolds couldn’t be triangulated at all. Yet Freedman’s and Casson’s work didn’t reveal whether triangulation is possible for all manifolds in five or more dimensions. That answer would have to wait another three decades, when Manolescu picked up the puzzle.
A Four-Dimensional Snag
Manolescu specializes in low-dimensional topology, which means that he works on problems of three- and four-dimensional manifolds. The question of whether manifolds in five or more dimensions can be triangulated would seem to lie outside his area of expertise. But in the 1970s, three mathematicians proved that solving the triangulation conjecture in higher dimensions was equivalent to answering a different question in lower dimensions. This transformation of one question into another is common in mathematics and can often provide a new perspective on a seemingly intractable situation. In 1994, when Andrew Wiles proved Fermat’s Last Theorem, what he actually solved was a different problem, the semistable case of the Taniyama-Shimura-Weil conjecture, which earlier had been shown to imply the proof to Fermat’s question.
Similarly, in the 1970s, a pair of mathematicians at the Institute for Advanced Study in Princeton, N.J. — Ronald J. Stern and David Galewski — and a third mathematician working independently, Takao Matumoto, “reduced” the triangulation conjecture from a question in high dimensions to one in low dimensions.
To see how it works on a conceptual level, first imagine a two-dimensional sphere and the two-dimensional triangles that need to be glued together in order to triangulate it. One way to glue the triangles is to start with the highest-dimensional part of their boundary — their one-dimensional edges — and move on to their next-highest-dimensional part — their zero-dimensional vertices.
Now consider, say, a seven-dimensional manifold. You’ll need seven-dimensional simplices to try and triangulate it. To begin, you might take the same approach you took with the triangles, first gluing together the highest-dimensional part of the boundaries of these simplices (six-dimensional “edges”) and working your way down from there.
What Galewski, Stern and Matumoto showed is that this gluing process goes quite well at first but snags on the border between dimensions four and three. The nature of this snag roughly boils down to a question about a topological space known as a “homology 3-sphere,” which forms on the boundary between these dimensions. And deciding that question required a new kind of invariant, one that Manolescu would eventually find in his work in Floer homology.
The Big Break
Floer homology is a mathematical toolkit developed in the 1980s by Andreas Floer, a brilliant young German mathematician who died in 1991 at the age of 34. It has turned out to be an incredibly successful way of thinking about manifolds, and it is now more a subfield of topology than a specific operation. Since Floer first proposed it as a way of working with three-dimensional manifolds, other mathematicians have created dozens of varieties of Floer homology, each suited to solving different kinds of problems. In the 1990s, Peter Kronheimer, Manolescu’s dissertation adviser at Harvard, and Tomasz Mrowka, a topologist at the Massachusetts Institute of Technology, combined equations that originated in quantum physics with Floer homology to construct a powerful invariant of three-dimensional manifolds. In his dissertation, Manolescu created a simplified version of their theory.
“Ciprian [created] a simple, less technical way to define this Floer homology, and because it’s less technical, it allows you to become more creative,” said Mrowka. “You don’t need to carry this big toolbox around to get the job done.”
Manolescu turned Floer homology into a lighter, nimbler instrument in his dissertation, but neither he nor anyone else was immediately sure what to do with it. So there it sat, an impressive piece of work with no clear application.
In the meantime, Casson and the Norwegian mathematician Kim Frøyshov had each come up with invariants that did part of the job of solving the triangulation conjecture. But neither advance was enough on its own. “You need two properties for the invariant, and Casson had one and Frøyshov had another,” Manolescu said.
Manolescu began to think about Casson’s and Frøyshov’s failed attempts in late 2012, and he had two important insights in quick succession. First, he realized that a major limitation of Frøyshov’s invariant was that it didn’t take advantage of a certain kind of symmetry known as Pin(2) symmetry. Next, he realized that his work on Floer homology eight years before was perfectly suited to incorporating that symmetry into the proof.
“There were just two [missing] ideas,” Manolescu said. “In retrospect they seem straightforward, but somehow they were missed.”
Once Manolescu understood the connection between his dissertation and the triangulation conjecture, he moved quickly. “I was very excited, and I wanted to write it down as fast as possible,” he said. “I was kind of working around the clock.” It took him a month to lay out his full refutation of the triangulation conjecture. He created a new invariant, which he named “beta,” and used it to create a proof by contradiction. Here’s how it works: As we have seen, the triangulation conjecture is equivalent to asking whether there exists a homology 3-sphere with certain characteristics. One characteristic is that the sphere has to have a certain property — a Rokhlin invariant of 1. Manolescu showed that when a homology 3-sphere has a Rokhlin invariant of 1, the value of beta has to be odd. At the same time, other necessary characteristics of these homology 3-spheres require beta to be even. Since beta cannot be both even and odd at the same time, these particular homology 3-spheres do not exist. Thus, the triangulation conjecture is false.
A New Set of Tools
On March 10, 2013, Manolescu posted a preprint of his paper to the online repository arXiv.org; the paper is currently under review at the Journal of the American Mathematical Society. Stern calls Manolescu’s proof “the best result in the last couple years” in four-dimensional topology. The finding has prompted speculation that Manolescu will win the Veblen Prize in Geometry, which is given every three years for outstanding work in geometry or topology. (Casson, Freedman, Kronheimer and Mrowka are all former winners.)
“Nobody, certainly not I, thought about using that version of Floer homology to solve the problem,” said Stern. “The ingeniousness of it was just the approach [Manolescu] took.” Stern added that he maintains an informal “bucket list” of problems he’d like to solve, and the triangulation conjecture was on it. “I would either like to have solved it or I’d like to know the answer,” he said, “and now I know the answer.”
But the more significant consequence of Manolescu’s proof is the way it elevates his version of Floer homology. “For whatever reason,” Mrowka said, “people haven’t picked it up as much as they should have.” Now, with the refutation of the triangulation conjecture, mathematicians are rushing to learn how to use Manolescu’s powerful tool.
Currently, topologists at the California Institute of Technology and the University of Texas, Austin, are running seminars on Manolescu’s thesis. Mrowka has two graduate students working on proving Manolescu’s results again and refining his methods for other uses. Manolescu’s techniques could be useful for answering questions in four-dimensional topology and other important topological sub-disciplines. No one knows exactly what they will end up being useful for.
“It seems hard to believe that having a wonderful new invariant isn’t going to have applications to problems that have been around in related areas,” said Mrowka. “But as to what, who knows? That’s what research is for.”
Correction: Due to an editing error, an earlier version of this article implied that Andrew Casson’s efforts to create an invariant that ultimately helped solve the triangulation conjecture occurred after Manolescu completed his Ph.D. In fact, the work occurred earlier. The article was revised on January 14, 2015, to eliminate the implication.