Too Small for Big Muscles, Tiny Animals Use Springs
Introduction
“Although Galileo demonstrated the contrary more than three hundred years ago, people still believe that if a flea were as large as a man it could jump a thousand feet into the air,” wrote the biologist J.B.S. Haldane in his delightful 1926 essay, On Being the Right Size. Galileo’s square-cube law had established that as objects get larger (by, say, some linear factor n), their surface area and volume increase far faster (by n2 and n3, respectively). The jumping muscles of a hypothetical six-foot flea could never keep pace with its scaled-up weight, Haldane explained.
The fact that surface area and volume increase faster than an object’s linear dimensions has profound and widespread implications for biology. All living things feed, breathe and get around, but the various mechanisms used for them rely on biophysics that works best within certain size domains. As organisms get larger or smaller, they need solutions appropriate to their scale.
An extensive literature has probed how organisms cope with the challenges of size in the decades since Haldane’s essay, but discoveries continue to be made. Recently, a paper in Science described and quantified the sometimes-explosive releases of power displayed by fleas, ants, mantis shrimp and other tiny creatures when they leap or strike with their claws or jaws. Lacking big muscles, they use the mechanical properties of their body’s structural materials like loaded springs. Evolution’s ingenuity in achieving such performance anticipated the designs that humans would eventually invent for their own tools and weapons — and is inspiring researchers and engineers today in their work on more capable robots.
Locomotion and Breathing
Classic studies in biomechanics research have explored the implications of size for organisms. Getting bigger and heavier, for instance, poses problems for an animal’s locomotion and posture.
“As you go from mouse to elephant, the amount of bone — the cross-sectional area that ultimately determines its strength — doesn’t increase as quickly as the mass of the animal,” explained Thomas Roberts, a biomechanics researcher at Brown University. To prevent their bones from breaking, he said, large animals adopt more upright, columnar postures that keep their body weight better distributed over the load-bearing parts of the bones. Think of a giraffe’s tall, mostly vertical structure.
Conversely, when a body scales down in size, its mass decreases faster than its cross-sectional area. Ants can easily carry several times their body mass because geometry makes their exoskeletons, and the muscles attached to them, disproportionately stronger than those of bigger creatures, Roberts said.
Size also matters for how animals breathe. Small things like insects rely on the diffusion of oxygen in the tracheal tubes that run through their bodies to directly service each cell’s metabolic needs, whereas large creatures need lungs or gills and active circulatory systems to deliver enough oxygen to their tissues (and, just as importantly, remove cells’ metabolic waste). In a seminal but controversial paper in 1997 in Science, Geoffrey West, now at the Santa Fe Institute, James Brown of the University of New Mexico and Brian Enquist of the University of Arizona argued that the geometry of these branching circulatory networks could explain a pattern known as Kleiber’s law: Metabolic rates from tiny flies to big blue whales appear to scale with mass raised to the 3/4 power. Even though the cells of a blue whale and of a fruit fly are roughly the same size, the whale’s cell has a lower metabolic rate. West, Brown and Enquist argued that the difference has to do with how the geometry of these branching oxygen and nutrient delivery systems scale with body size.
Just as the diffusion of oxygen into tracheal tubes works for insect respiration but not for larger animals, other biological design strategies work well at certain sizes yet become unwieldy at larger or smaller ones. Ants, lobsters and many other invertebrates support their bodies with chitinous exoskeletons, but for much larger creatures, bony internal skeletons have been a better solution: Large exoskeletons would become either too constraining and heavy or too weak and prone to breakage.
Muscles, Springs and Latches
Muscular power is another area in which scale matters. Nearly all multicellular animals, except for simple ones like sponges, depend on muscles to move, and all their muscles use some form of contracting fibers in which filaments of the proteins actin and myosin pull past one another to generate force. Muscles can generate approximately 100 to 300 watts per kilogram of power (power is the amount of energy transferred per unit of time).
But small animals face problems that muscle power alone can’t solve. When running, for example, the legs of small animals are in contact with the ground for only very brief moments during each step, which constrains how much energy each stride can release.
To address this problem, many small animals use flexible structures in their bodies as springs that they can cock and release like an archer’s bow. The spring enables the small animal to store energy slowly and then release it all at once, thus amplifying its power.
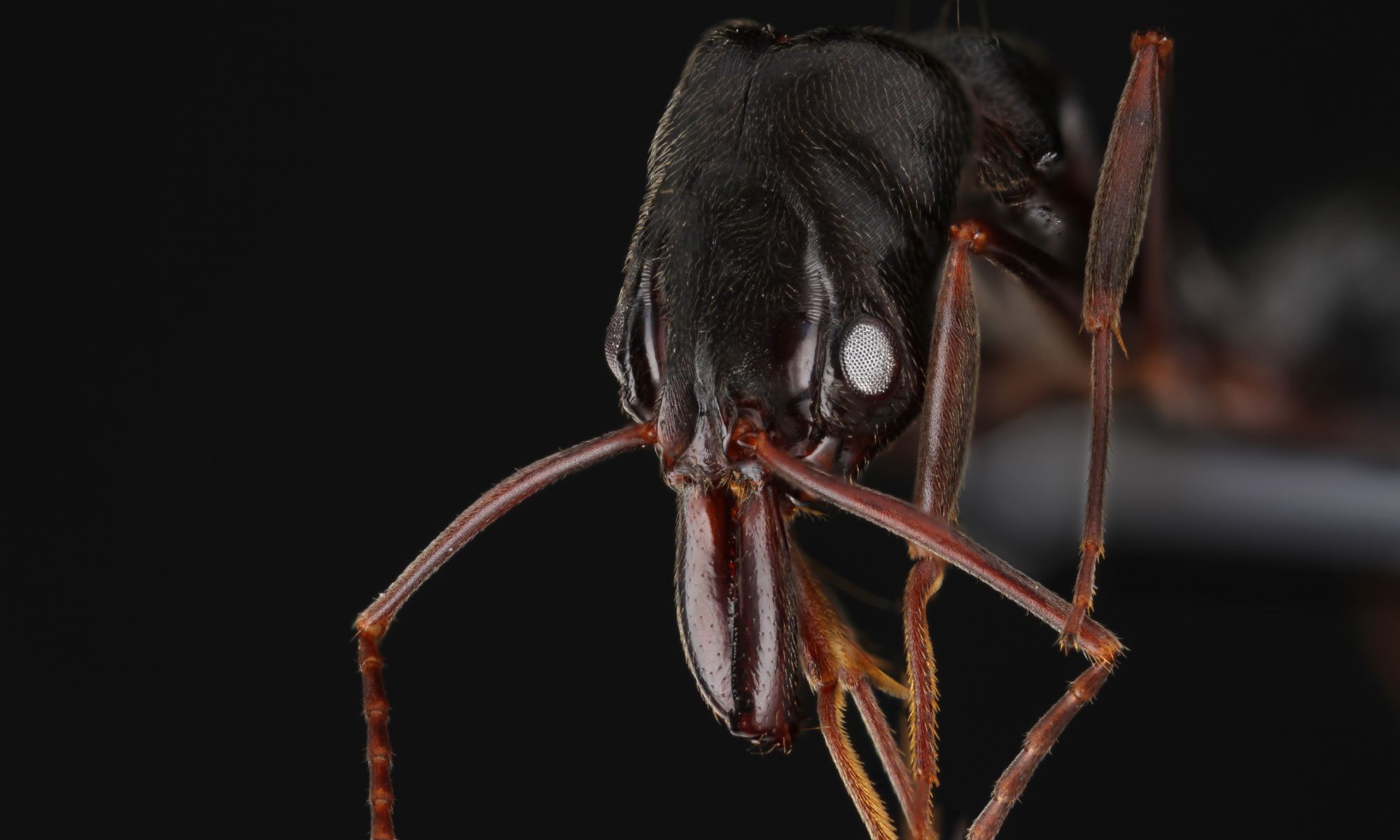
The trap-jaw ant uses a latch-and-spring mechanism to power the bite of its imposing mandibles.
The insect called a froghopper, for instance, is a prodigious jumper even though its legs are much shorter than those of crickets and locusts. Its secret is that it uses its leg muscles to bend the chitinous exoskeleton encasing its thorax, which functions as a spring or bow. A latch holds the stored energy in the bow — as much as 65,000 watts per kilogram. When the latch releases, the exoskeleton springs back to its original shape, unleashing a powerful jump that can hurl the froghopper 100 times its body length.
This springy solution works for more than jumping. The head of the trap-jaw ant is covered with a chitinous exoskeleton that muscles inside the head can bend like an archer’s bow. When the bow releases, it yields as much as 200,000 watts per kilogram, and the mandibles snap closed on unlucky prey at speeds of more than 140 miles per hour. When faced with a predator, the trap-jaw ant can also snap its mandibles against the ground with enough power to propel itself into the air, quickly escaping to safety.
The use of springs is not restricted to insects. When a frog crouches, its leg muscles stretch its long Achilles tendon like a spring and store energy in it. The release of that built-up tension propels the frog’s leap, said Christopher Richards, a paleo-robotics researcher at the Royal Veterinary College at the University of London, who is using a combination of robotics, modeling and anatomy to understand how extinct frogs with diverse pelvic shapes and leg proportions used to jump.
The latch that the frog uses to release the stored power, however, remains a subject of intense debate: “That’s the million-dollar question,” Richards said. “Nobody has found an anatomical latch in frogs. To my knowledge, nobody has found a latch in a vertebrate animal.”
The latches have been figured out for only a handful of insect and crustacean systems. Latches are harder to find than springs because the latch mechanism is usually inside the animal’s body, as opposed to the easily accessible springs made of crustaceans’ outer cuticle or insects’ exoskeleton. Unfortunately, dissection destroys the delicate spring-and-latch systems, making it difficult to determine how they work in living organisms, explained Gregory Sutton, a biomechanics researcher and engineer at the University of Bristol. Usually, researchers end up inferring the existence of a latch from the abrupt release of power from an identified spring. “Something has to switch the system from a mode where the muscles are stretching the springs to a mode where the spring is recoiling and powering, powering that huge motion,” Sutton said.
The first latch to be discovered was the one in grasshoppers, which was described in 1977 by William James Heitler of the University of St Andrews in Scotland. Grasshoppers use what is called a geometric latch: The opposing muscles of the leg work in pairs. First, when the leg is fully flexed, the big muscle of the leg loads the spring while the smaller muscle stabilizes the knee joint. By slightly moving the joint, the small muscle then causes a change in the moment arms of the two muscles and triggers the joint to rotate out of control, setting off the jump.
Moving Past Idealized Springs
Although the spring-and-latch systems that small creatures use have been studied for decades, there has been a serious weakness in scientists’ understanding of them. “Until now we’ve largely treated spring systems as though they’re unlimited in their ability to store energy and release power,” Roberts explained. That’s an acceptable assumption when the mass of the springs involved are negligible relative to body mass. But a mechanical constraint on any spring is that, when it is unloading energy, it has to move its own mass, which inevitably reduces the force output of the spring in a way that is proportional to the velocity of the moving object, Roberts said. For small systems, the mass of the spring frequently becomes an appreciable part of the total mass and has to be taken into account.
Latches were also modeled in an idealized fashion, as if they released springs instantaneously. But the speed at which a latch releases the energy stored in a spring turns out to be important: It determines how quickly the load on the spring can accelerate, Richards said. If animals are limited in how quickly they can release the latches in their biomechanical systems, their performance would also be limited.
In an April 26 Science paper, an interdisciplinary team of engineers, physicists, physiologists, biomechanics researchers and materials scientists presented a new theoretical model that makes explicit use of these real (rather than idealized) properties of muscles, latches and springs, and mathematically describes how the properties of these components must be tuned to one another to optimize performance.
“What to me is the major breakthrough here is, now we can follow the path of power,” said Sheila Patek, the senior author of the paper and an associate professor at Duke University who investigates the mechanics of movement. “We can see which systems should be governed by a spring and a latch and which don’t benefit from that, and we can get a much better handle on classic scaling [problems] in biology that haven’t made sense before this.”
Mantis Shrimp and Robots
Patek has been studying the behavior and biomechanics of mantis shrimp, also known as thumb-splitters, since 2002. They are small crustaceans with hammerlike claws the size of toothpicks that they use to break open snail shells (or to slice open the finger of an unwary human handler).
“We [humans] could seriously be hitting a snail shell with two toothpicks for the rest of our lives and never break a snail shell, right?” Patek said. Yet as she and her colleagues showed, the mantis shrimp can do it because of the latch-and-spring system powering its claws. A muscle loads resistance into the chitinous exoskeleton spring, which is held in place with a yet-uncharacterized latch. Then the latch releases, and the spring accelerates the hammer outward at speeds of up to 30 meters per second. “Their strike is similar to the acceleration of a bullet in the [barrel] of a gun (105 m/s2),” Patek clarified by email.
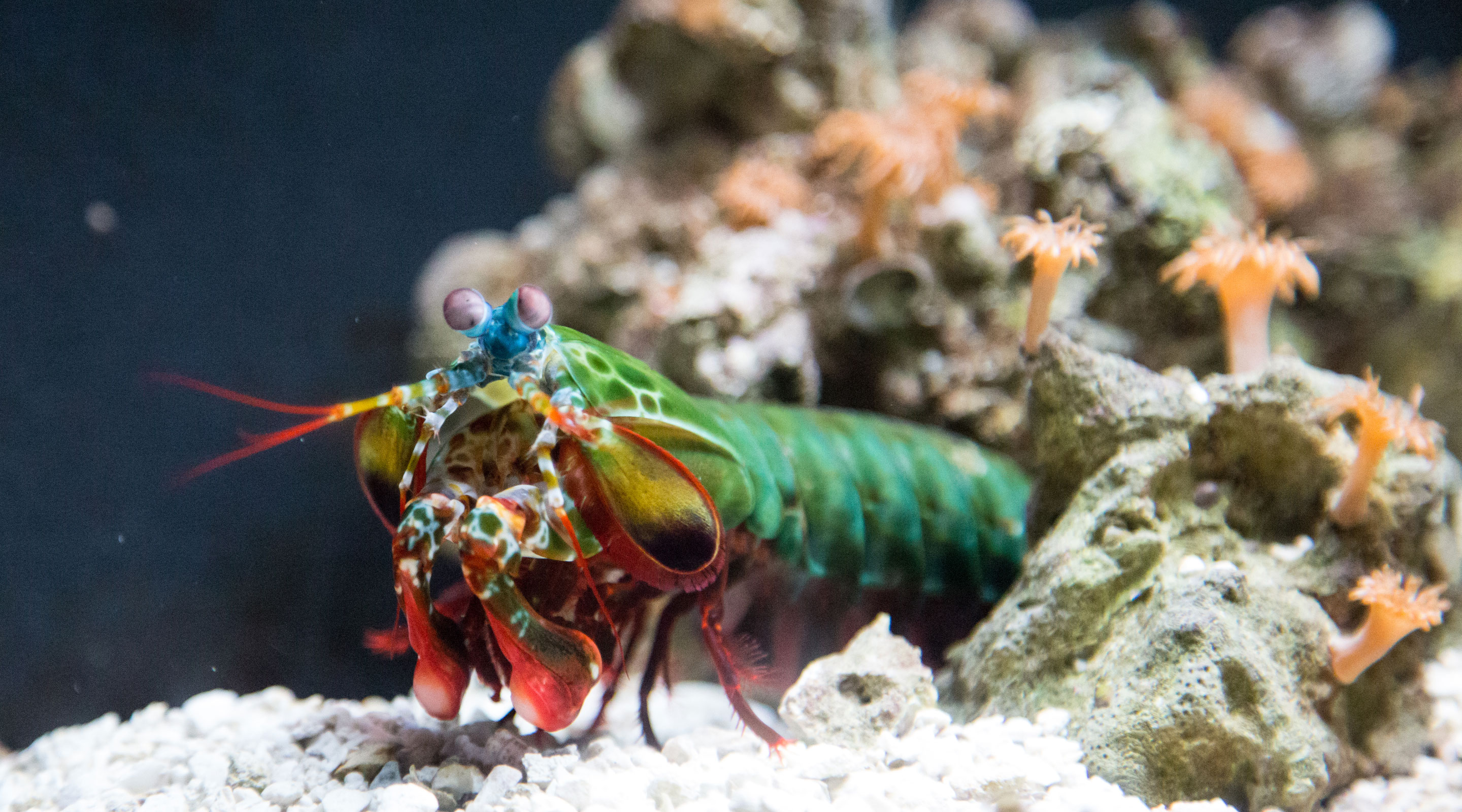
Mantis shrimps, or thumb-splitters, use a latch-and-spring mechanism to accelerate their toothpick-size claws to bulletlike speeds, which enables them to punch through snail shells.
Because biologists have been so focused on what muscles can and can’t do, they have largely neglected the spring itself, Patek explained. “We need to look at how that material behaves when it’s pushing the hammer, and then of course, what the latch is doing,” Patek said. Now that the team has laid out these questions formally in their paper, and there’s a vocabulary with which to talk about the problem, they and others are now exploring the diversity of latches and springs across a variety of biological and engineered systems.
For instance, another co-author on the Science paper, the mechanical engineer Sarah Bergbreiter of the University of Maryland, College Park, designs microrobots for a variety of applications including search and rescue (for going through the rubble of a collapsed building after an earthquake, for example), space exploration and medical uses (a tiny robot could be swallowed in a pill to grab a biopsy of the intestinal tract). All these small robots will need to move, jump or punch holes with sufficient power. The idea is to use some of the lessons learned from nature to improve small robotic design, explained the lead author, Mark Ilton, a physicist and materials scientist at the University of Massachusetts Amherst.
Historically, biological systems have outperformed engineered ones, and this new model may pave the way for robots to catch up, according to Suzanne Cox, a co-author and biologist at Pennsylvania State University. Biology is very diverse in terms of organisms and habitats, but it doesn’t have a huge toolbox of materials, Patek said. Over evolutionary history, just four polymeric materials — cellulose (in plants), chitin, collagen and resilin (an elastic protein found in insects) — have been organized or shaped in a wealth of ways to maximize the power output of mechanical systems in living things, she explained.
On the engineering polymer sciences side, it’s the complete opposite. Materials scientists have a big set of materials in their toolbox, Patek said, but they generally have not explored much about how to interconnect these materials or to shape the materials for optimum performance. “The framework that we’ve developed basically allows you to explore the design space in these jumping systems,” Bergbreiter added.
The Right Size for the Right Trick
Although springs are useful across biology, the advantages and drawbacks of motors (for robots) and muscle (for animals) versus spring-and-latch systems are going to balance out differently depending on size. In general, springs and latches are more advantageous at smaller sizes; their advantages become less clear as the loaded masses increase and inertia makes springs less effective.
“When you’re large, the choice that you have between a spring and a motor is not as clear-cut,” said Gregory Sawicki, a biomechanical engineer at the Georgia Institute of Technology. “If I’m large, I can get basically the same performance out of a motor as a spring. Therefore, I might choose a motor because the motor is more versatile.” In particular, a motor or muscle gives the user more control. “If you want to control the energy flow more carefully, these ballistic spring systems are difficult to deal with,” he explained.
Another drawback of springs is that organisms that push spring-and-latch systems to their performance limits have to worry about breakage. Patek noted that for mantis shrimp, the problem of avoiding self-destruction is severe. “They have to get the energy out of their body [and] try not to have it go back in and tear the muscles in the leg,” she said.
Mantis shrimp, trap-jaw ants, froghoppers and other small things also have to worry about breaking their springs. These animals have only one set of springs and latches to use in their limbs throughout their lives. Breaking a spring could be deadly.
One secret to their trick of avoiding breakage appears to be that rubbery protein called resilin, which insects have in their wing hinges and tracheal tubes. Resilin is also found in the cuticular springs, where it is configured in thin layers beneath the chitin. In fact, the layering of chitin and resilin resembles the design of archers’ composite bows from hundreds of years ago, such as those made of horn and wood, or leather and wood. The composite bow can “resist damage and be used repeatedly without losing its mechanical properties,” explained Sutton. The layering of different materials prevents any tiny cracks from spreading, thus limiting damage and giving the animal a chance to repair those cracks before they become catastrophic. The design of composite bows was described in 1545 by Roger Ascham, a tutor to Queen Elizabeth I and a Cambridge scholar, noted Sutton, whose historian friend first spotted the similarities between the design of ancient bows and the microstructure of insect exoskeletons.
Breakage becomes more of a problem as organisms get smaller. Consequently, there appears to be a sweet spot in body size — neither too big nor too small — at which animals can make optimal use of latches and springs. Haldane, in his essay, had discussed the implications of the square-cube law and relative muscle efficiency for hypothetical giant fleas. But in light of Patek and colleagues’ work, the real limitation on fleas’ jumping abilities seems to be that their tiny springs — which are far smaller than the robust ones of mantis shrimp, for example — can tolerate only so much stress.
“It’s very hard to build a spring that is incredibly small without it breaking,” explained Sutton. “That’s why fleas are not actually terribly good jumpers — because their springs just aren’t big enough to handle the forces involved.”
This article was reprinted on ScientificAmerican.com.