Touch, Our Most Complex Sense, Is a Landscape of Cellular Sensors
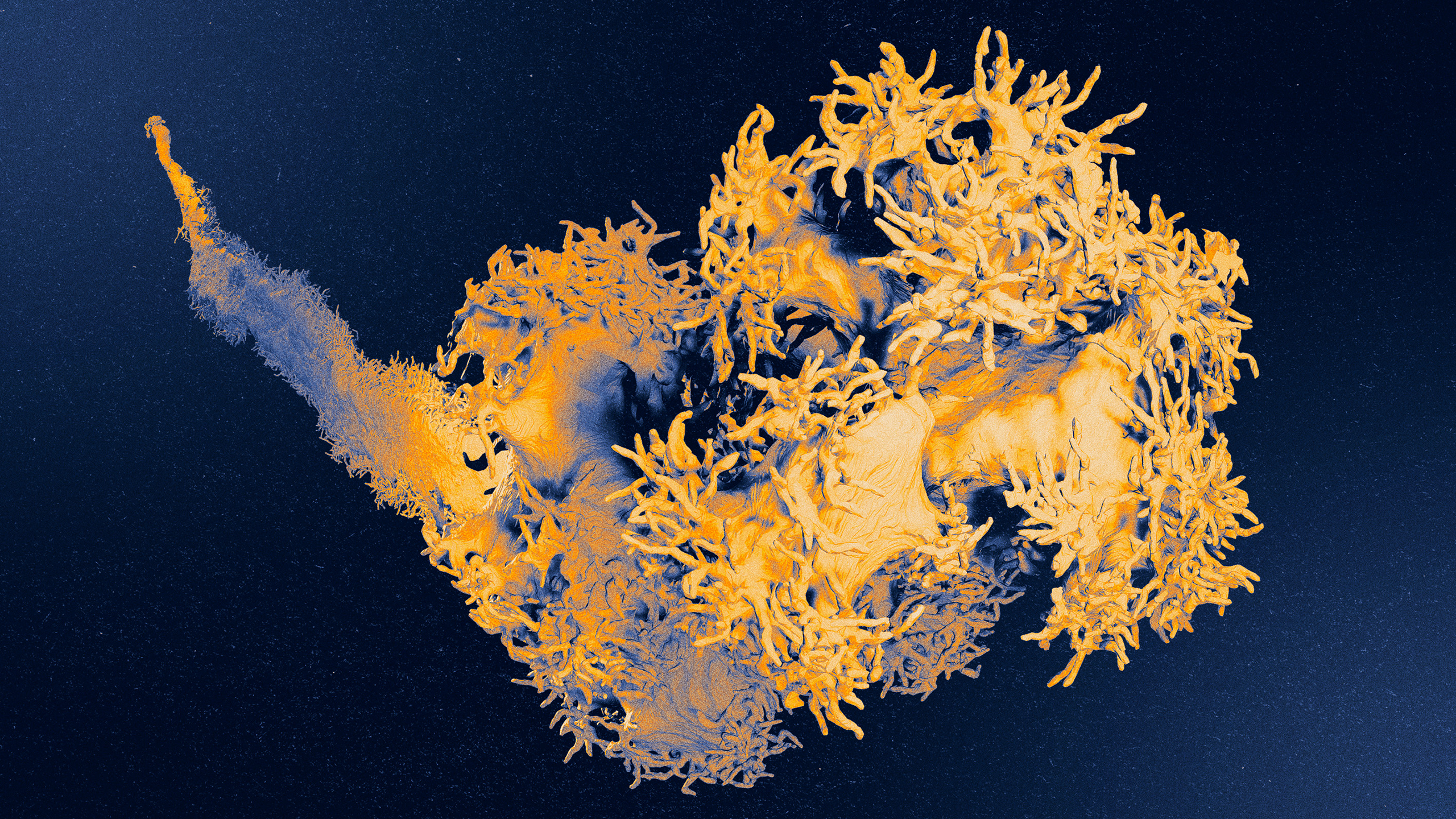
Buried deep in the skin, the Pacinian corpuscle is a type of touch sensor that picks up geologic vibrations. When the fingerlike protrusions on the neuron’s axon vibrate and stretch, the cell is activated, which we experience as rumbling.
Courtesy of David Ginty
Like many proud parents, David Ginty has decorated his office with pictures of his genetic creations. There’s the prickly one sporting a spiked collar and the wannabe cowboy twirling a lasso. There’s the dramatic one, always reacting to the slightest provocation; the observant one that notices every detail; the golden child Ginty loves to boast about. “They’re like a family,” he said. “Each one has its own quirks and individual characteristics.”
They’re not really a family and, anyway, they’re not his children. They have evolved over millions of years to give humans and other mammals an interface with the physical world around us. But Ginty, who heads the neurobiology department at Harvard Medical School, has been studying this quirky cast of characters — the sensory neurons of touch — for more than two decades, and has gotten to know them better than anyone else ever has. He has learned their electrical language and what forces excite them, and charted their intricate paths into the skin and up to the brain. And, through feats of genetic engineering and chemical labeling, he has produced the colorful portraits on his walls.
“David Ginty is the emperor of touch,” said Alexander Chesler, a sensory neuroscientist at the National Institutes of Health.
“You look at his publication list and you go, ‘Oh my God,’” said David Hughes, a neuroanatomist at the University of Glasgow. “He’s so massively productive, and all his papers are published in the very highest-impact journals.”
Beyond the technical breakthroughs and the discoveries fit for biology textbooks, it’s the images that stick in his colleagues’ minds. They’re otherworldly, like deep-sea creatures — not at all what you might imagine neurons could look like. These strangely shaped cells are the reason why the experience of touch is so rich and multifaceted — why a buzzing cell phone feels different from a warm breeze or a lover’s caress, from raindrops or a mother’s kiss. To realize that your body is covered in them — that they are a part of you — takes your breath away.
“Each one of these neurons tells a story,” Ginty said. “Each one has a structure that is unique and responds to different things. It’s all about form underlying function. That’s where the beauty is.”
The Touch Landscape
Scientists and philosophers have been enamored with touch for centuries. Aristotle believed that humans’ tactile abilities eclipsed those of all other species, which partially accounted for our superior intelligence. However, we now know that creatures as diverse as sea lions, spiders and star-nosed moles can feel features of the physical world that are imperceptible to us. Yet Aristotle wasn’t wrong to view touch as exceptional.
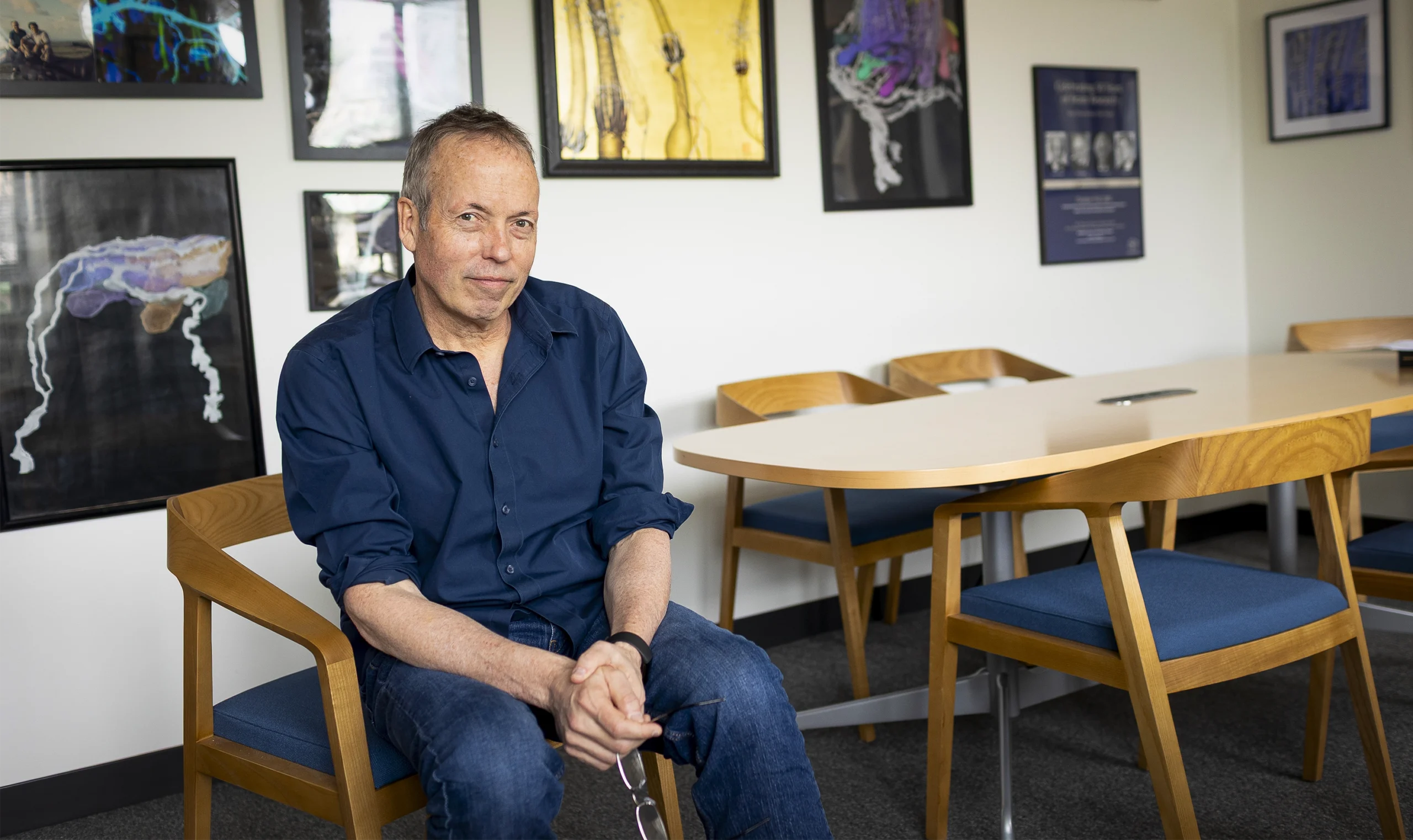
In the office of David Ginty, the head of the neurobiology department at Harvard Medical School, the walls are covered in images of the diverse touch neurons that innervate skin. “Each one of these neurons tells a story,” he said.
Katherine Taylor for Quanta Magazine
Of all the senses, the somatosensory system is the most complex, and therefore touch, some researchers argue, is the most difficult to study. Vision and hearing, for instance, are confined to the retina and the cochlea — parts the size of a postage stamp and a pea, respectively. Touch, however, is diffuse: The neurons that relay touch signals reside in clusters outside the spinal cord, from which they extend a vast web of axon fibers, like jellyfish tentacles, into the skin and internal organs. Each axon forms an ending just beneath the skin’s surface; the different types of endings are mechanisms for picking up and interpreting the variety of touch sensations.
While our eyes and ears each process information related to light or sound, touch concerns a smorgasbord of stimuli, including pokes, pulls, puffs, caresses and vibrations, as well as a range of temperatures and chemicals, such as capsaicin in chili peppers or menthol in mint. From these inputs arise perceptions of pressure, pain, itchiness, softness and hardness, warmth and cold, and the awareness of the body in space.
But how? Nineteenth-century anatomists provided the first clue. Peering at cadaver skin under a microscope, they noticed strange structures nestled in the tissue. Some were flat as pancakes, others looked like onions, spindles or balls of twine. Because these structures appeared to involve nerves, the anatomists concluded they were touch sensors.
In the 20th century, physiologists proved them right. Using a tiny wire electrode, they could listen in on the electrical chatter of a single neuron. Then, by testing out all manner of touching — say, by jabbing a human or animal’s skin with a pin or a pencil eraser — they could determine which stimuli the neuron responded to and where on the body it detected them. In animal subjects, they could dissect the receptive patch of skin to see what was there and, in this way, match the neuron to the structure its axon formed where it terminated in the skin.
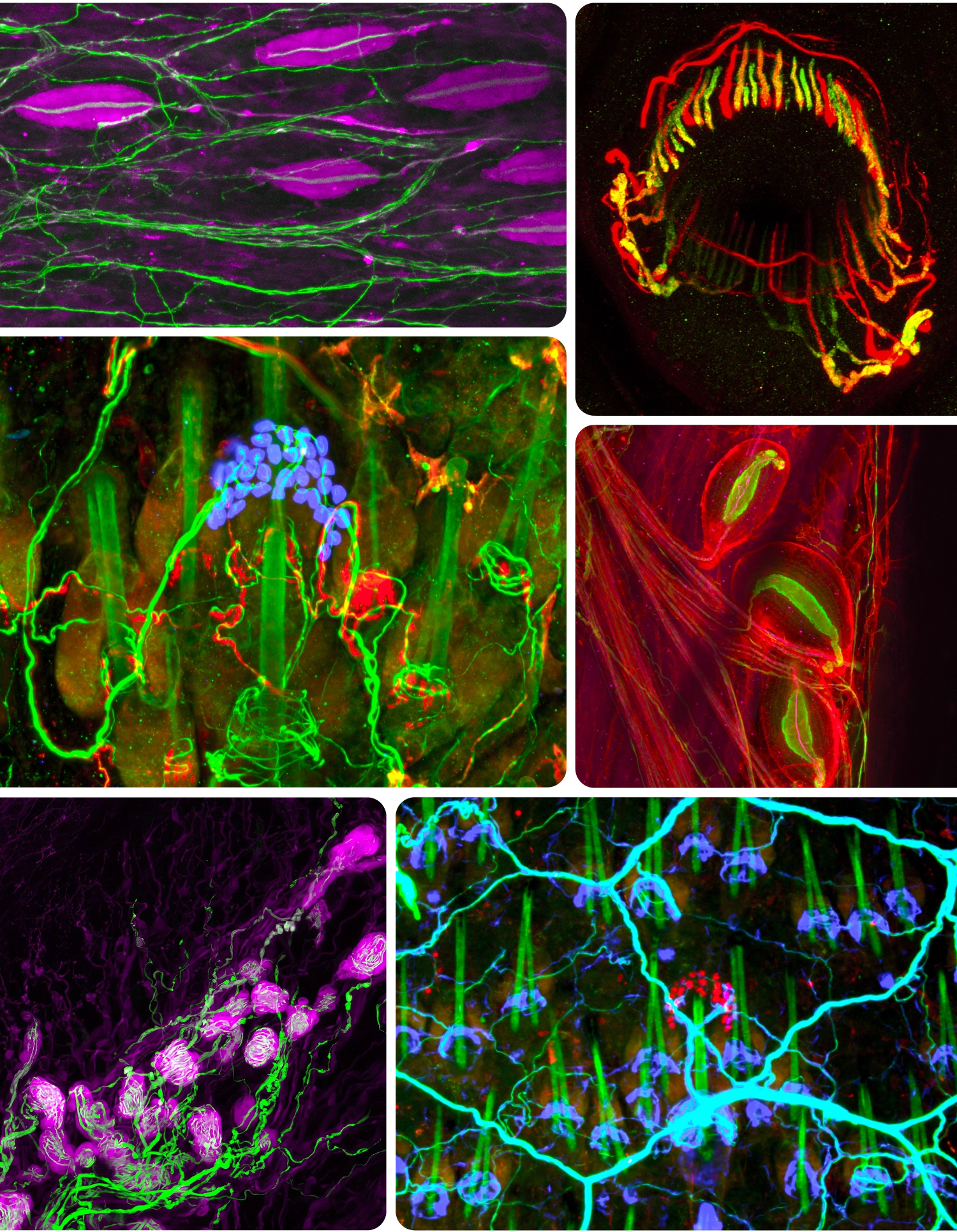
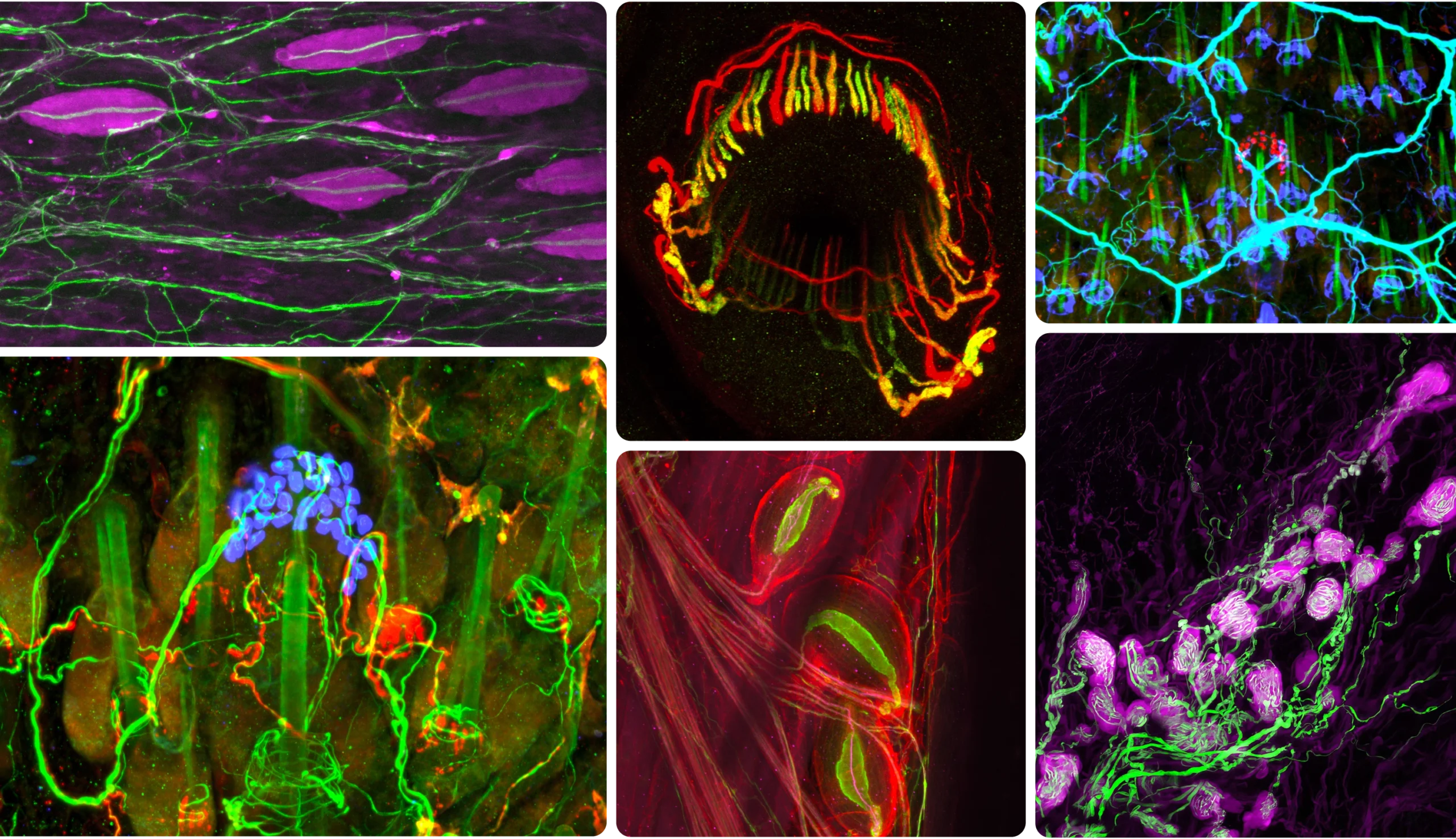
Using genetic tools and antibody stains, Ginty’s lab has created fluorescent portraits of touch neurons. Clockwise from upper left: Pacinian corpuscles sense geologic rumbling; lanceolate endings encircle hairs to detect deflection; three Pacinian corpuscles near a bone; Merkel cells (red), here encircling a hair shaft (green), discern gentle indentation; Krause corpuscles sense vibrations of sexual touch; Merkel cells (blue).
Courtesy of David Ginty
Using genetic tools and antibody stains, Ginty’s lab has created fluorescent portraits of touch neurons. Clockwise from upper left: Pacinian corpuscles sense geologic rumbling; lanceolate endings encircle hairs to detect deflection; Merkel cells (red) encircling a hair shaft (green) discern gentle indentation; Krause corpuscles sense vibrations of sexual touch; three Pacinian corpuscles near a bone; Merkel cells (blue).
Courtesy of David Ginty
Such nerve endings turned out to be remarkably specialized. Near the skin’s surface, the flat variety, called a Merkel cell complex, responds to gentle indentation. Merkel cells abound in your lips and fingertips, allowing you to discern form and texture. Your fingers are also packed with coiled nerve endings called Meissner corpuscles, which wrap around support cells in a bulbous tangle. These sensors pick up the faint, minuscule vibrations generated by the slight slipping of an object against your hand as you grip it, enabling you to use tools with precision. Deeper in the skin dwell the onionlike Pacinian corpuscles, which detect rumblings in the earth, and the spindle-shaped Ruffini endings, which convey skin stretching.
From their electrode recordings, the physiologists identified more than 15 varieties of touch neurons based on the size of their fibers and their firing patterns and speed. However, this emerging picture of the touch landscape was far from complete. Where did all these neurons go, and what, exactly, did they do there? Nerve endings such as Merkel complexes were easy to spot because they were large — roughly the size of a grain of salt. But most endings were too small to make out with the techniques of the time. Using tissue stains, researchers could trace their paths in the skin, but it was impossible to tell them apart.
“It was a jungle,” Ginty said. “There are probably between 12 and 15 neuron types that innervate the same region of skin, but you couldn’t distinguish them because it was just a web of axons.” It would take a half-century for the right convergence of technology and ingenuity to untangle it.
A Hairy Problem
Ginty is in his early 60s, but you wouldn’t guess it from talking to him. He describes the neurons that innervate skin with childlike enthusiasm, as if he’s just discovered them for the first time — when, really, he has thought about them every day since he started his lab at Johns Hopkins University in 1995.
“He’s actually very goofy and lighthearted,” said Rachel Wolfson, one of Ginty’s former postdocs who now runs her own lab at Harvard Medical School. “He’s often whistling to himself.” That’s not to say he’s absent-minded — quite the opposite. He focuses his curiosity and sense of wonder on his work, which keeps his attention on the most important questions. Bring him a problem, and he’ll devise the perfect experiment to solve it. “He has an amazing clarity of thought,” Wolfson said.
The problems began early. When Ginty decided to venture into the field of touch neuroscience, he wanted to understand how sensory neurons develop. He soon realized, though, that he had no idea what the immature neurons he was studying developed into — what circuits and end structures they formed in a mature animal. To find out, he would need a way to visualize each type of neuron individually.
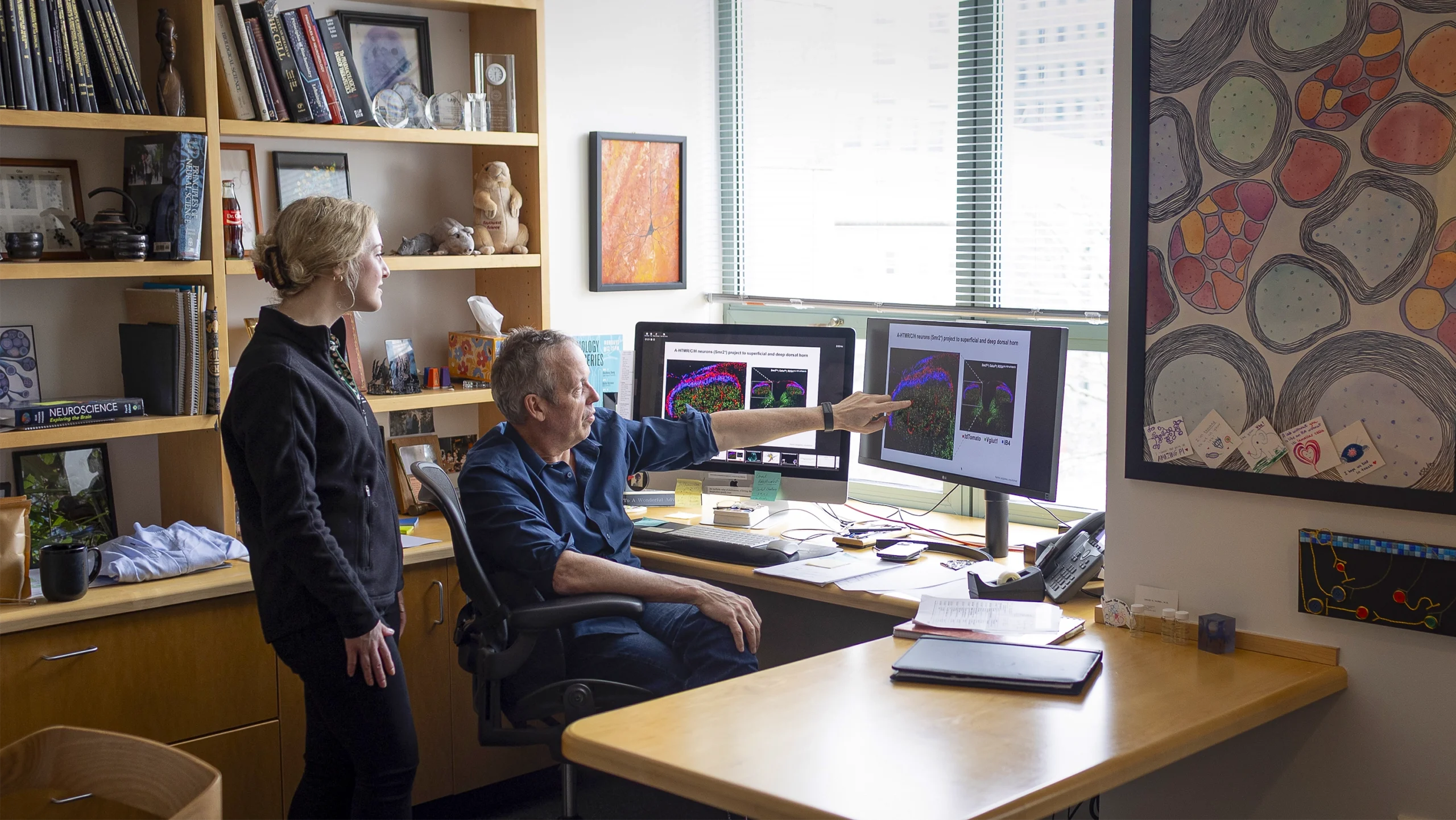
Ginty discusses sensory neurons with Zoe Sarafis, a graduate student in his lab.
Katherine Taylor for Quanta Magazine
Genetic technologies soon gave him a way to do so. During the first decade of his studies of neuronal development, Ginty amassed a library of genes specific to different touch neuron types. Around 2007, he began genetically engineering mice in which these genes could be used to control particular populations of touch neurons. In each new mouse breed, called a line, researchers could manipulate a single type of touch neuron in all sorts of ways, such as by marking it with chemical labels to make it fluoresce, or by turning it on and off with light.
“The amount of mouse lines that were generated in David Ginty’s lab is extraordinary,” Chesler said. “He literally developed dozens of lines” and then distributed them “like Johnny Appleseed” to other researchers.
Their impact was like hearing a symphony and then suddenly being able to see and play the instruments, Chesler said. Each mouse line shone a spotlight on a different section — the strings, the winds, the brass, the percussion. “You could pick up a violin and see how it works,” he said, “or you could change the tuning or alter the instrument in some way to see how it affects the sound of the music.”
One of the first things Ginty did with his new mouse lines was explore the subterranean world of hairy skin. Non-hairy, or glabrous, skin had been relatively well studied because it houses high densities of the familiar touch players, including Merkel complexes and Meissner corpuscles. Little was known, however, about the neural structures that let you feel the bend or pluck of a hair.
Although we tend to think of skin as mostly smooth, only the palms, the soles of the feet, the lips, the nipples and portions of the genitals are truly hairless. The rest of the body is blanketed in soft, pale hairs called vellus hairs, which wick sweat to keep us cool, and thicker, more visible guard hairs, which protect the skin against damage from rubbing and scratching. As Ginty observed, each type of hair is also a touch sensor.
Using their mouse lines and other genetic tricks, his team added fluorescent tags to three populations of sensory neurons known to respond to gentle touch, but whose axon endings were still obscure. Under a microscope, the neurons lit up like neon signs, revealing their endings in the mouse’s hairy back in brilliant red and green. The researchers saw comblike projections that wrapped around hair follicles like crowns. They named the projections “lanceolate endings” after the crowns’ tapered points.
Amazingly, all three neuron populations formed lanceolate endings, but each was associated with a different type of hair. Mice have three hair types: guard hairs as well as awl and zigzag hairs, which are akin to vellus hairs in humans. Ginty’s team showed that thick, fast-conducting neurons encircle the follicles of guard and awl hairs, allowing the mice to quickly discriminate where and how they’re being touched. Meanwhile, thinner neurons that conduct signals at lower speeds target awl and zigzag hairs. Ginty later found that these neurons form one-sided crowns — more like tiaras — which convey when a hair is brushed or pulled in one direction or another. Awl and zigzag hairs are also the targets of fine, slow-conducting neurons, which were once thought to evoke tickles but have since been implicated in pleasurable touch sensations. For this reason, they are sometimes referred to as caress sensors. (These neurons also cause dogs to shake when they’re wet, Ginty’s lab recently discovered.)
The team’s findings, published in 2011, revealed how hair can give rise to a multitude of touch sensations. “It was a real tour de force,” said Diana Bautista, a neuroscientist at the University of California, Berkeley, who was not involved with the work. “The complexity of the system — how these different neuron subtypes innervate the skin in this beautiful and organized way — was mind-blowing. And the images were incredible,” she said — like seeing the first pictures of Earth from space.
Good Vibrations
Ginty went on to map the landscape of the skin’s touch sensors in ever-greater detail. In a 2015 study, his lab (by then relocated to Harvard Medical School) discovered that another type of fast-signaling neuron ensnares hair follicles like lassos. The physiologists who had first described these neurons in the 1960s believed they were pain sensors. But Ginty found that they also sense light rubbing over a large area of hairy skin, like the back of a hand, suggesting that these neurons — which form what he calls “circumferential endings” — are in fact specialists in the gentle stroke. They detect this broad movement by sprouting as many as 180 arms, each one lassoing a different hair follicle. “They’re spectacular neurons,” he said.
More recently, he has delved into the mystery of sexual touch. In addition to discovering Merkel cells and their ilk, the early anatomists stumbled upon curious structures in external genitalia that resembled the bulb-shaped Meissner corpuscles in hands. These structures, called Krause corpuscles, are also found in the lips, nipples and anus, and, oddly, in the eyeballs and finger joints. Scientists presumed they played a role in sex, but it wasn’t clear what. Some speculated that they were heat sensors.
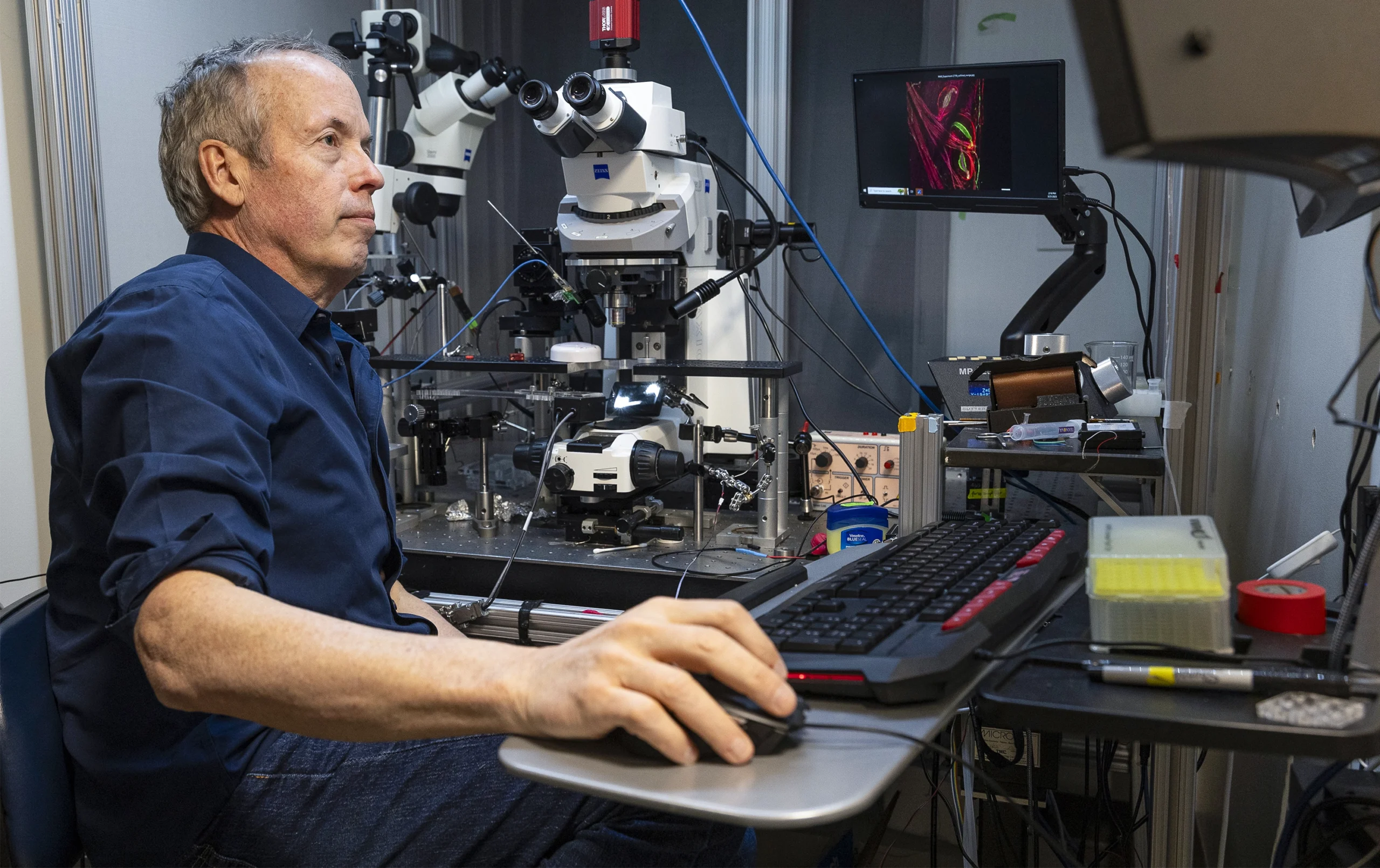
Ginty genetically engineered mice to have fluorescent markers on different touch neuron genes, letting him create portraits of the cells using confocal microscopy.
Katherine Taylor for Quanta Magazine
In a study published in June 2024, Ginty and his collaborators found that Krause corpuscles are composed of axon endings from two types of fast-conducting neurons. By activating or removing these neurons in mice, the team showed that they trigger sexual arousal. Like Meissner corpuscles, Krause corpuscles detect vibration. “‘Why vibration?’ you might ask,” Ginty said. “Because when skin glides across skin, it creates micro-vibrations. These guys are really good at picking those up.” Krause corpuscles are most sensitive to frequencies around 40 to 80 hertz, which is precisely the range of vibrating sex toys.
Ginty’s all-time favorite touch neuron, however, is the Pacinian neuron, whose axon forms the onion-shaped Pacinian corpuscle. In humans and other primates, Pacinian corpuscles lie deep in the underlayers of both glabrous and hairy skin and concentrate near joints. (In mice, they primarily reside near the wrist, ankle and digit bones.) Like Meissner and Krause corpuscles, these sensors are tuned to vibration, but at much higher frequencies — as high as 1,000 hertz. “What the hell vibrates in that range?” Ginty recalled wondering. The rumble of a train? The reverberation of a cello? The drum of a rainstorm? He knew that an elephant’s feet and trunk, which can detect the footsteps and calls of other elephants from miles away, are full of Pacinian corpuscles. Perhaps, he thought, these nerve endings are seismic.
To test this theory, Ginty’s team recorded electrical signals from Pacinian neurons in awake mice. When the researchers pounded on the ground beside the mice, the neurons fired. When they pounded several meters away, the neurons fired again. Even patting the ground faintly with a finger caused them to spike. “These neurons are unbelievably sensitive to environmental vibrations,” Ginty said. Even more remarkably, when the researchers traced the neurons’ path into the brain, they saw that the signals did not follow the usual route for touch stimuli — through the brainstem to the thalamus and, ultimately, the somatosensory cortex. Instead, as the team reported in 2024, most Pacinian neurons projected to the inferior colliculus, where the brain processes sound.
Ginty imagines Pacinian corpuscles as “little cochlear implants all over the body.” When they are activated, his team also discovered, the brain responds to sounds more strongly. “The mechanical vibrations make auditory cues more salient,” he said. This could explain why often, when we listen to music, we not only feel it in our bodies but also perceive the experience as profound.
Dizzying Diversity
Over the past five years, Ginty and other scientists have analyzed the genetics of thousands of individual touch neurons. Sorting these cells according to the genes they express, Ginty’s team has so far come up with 18 distinct types, maybe more — it’s hard to tell, given the limited resolution of their sorting tools. That total includes the six or seven gentle-touch neurons on which Ginty’s research has primarily focused, as well as six neurons for stronger mechanical stimuli (some of which also respond to temperature and chemical irritants), one neuron for painful heat, one for cold, three or more for sensing body position, and a few whose functions are unknown.
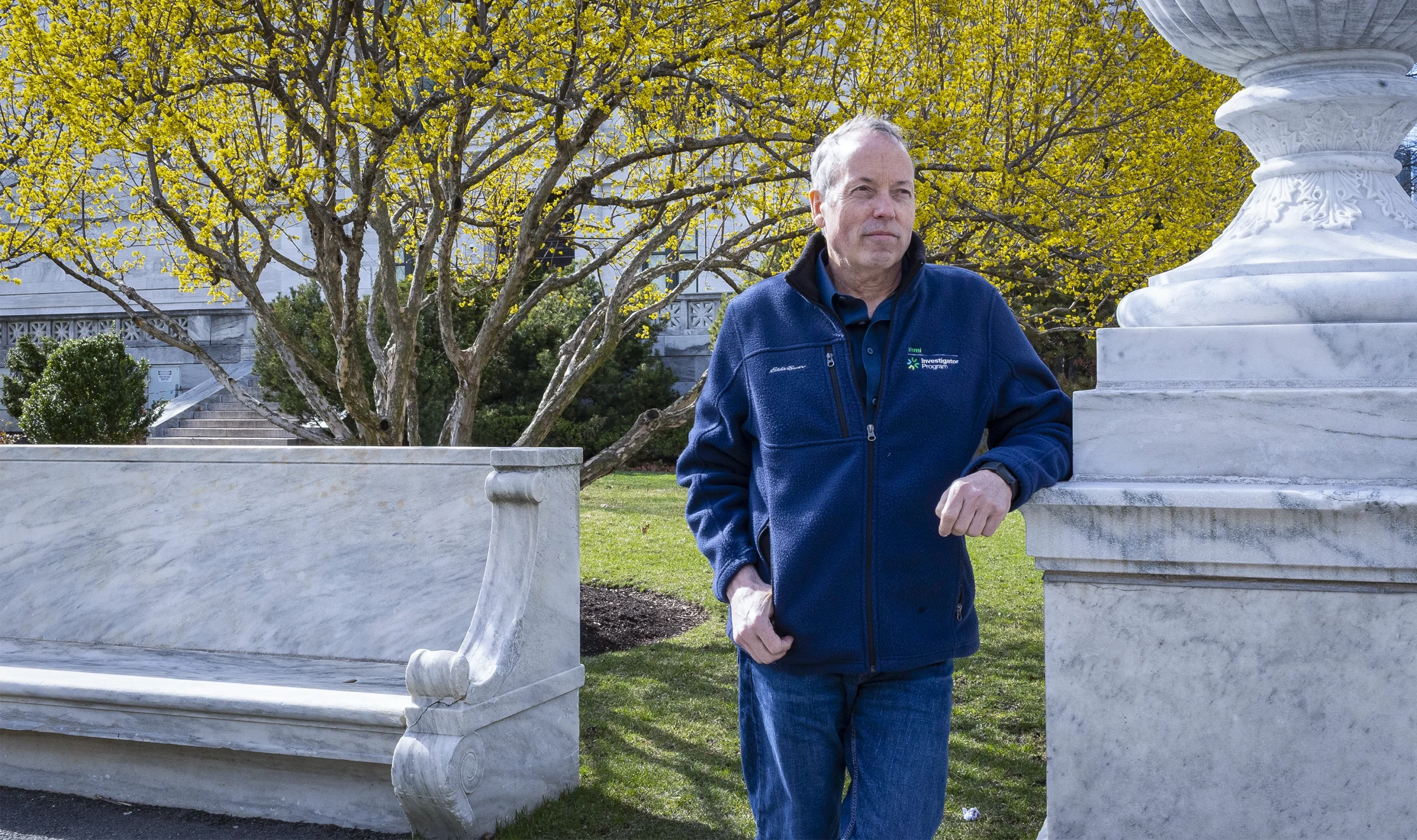
More than anything, awe motivates Ginty to map the skin’s landscape of touch neurons.
Katherine Taylor for Quanta Magazine
As more touch neurons are analyzed, the count will likely increase. And each genetically distinct type can be further subdivided based on its axon endings. Genetically identical neurons that form Meissner corpuscles for picking up vibrations in glabrous skin, for example, also form lanceolate endings for detecting hair movement in hairy skin. In a 2023 study, Ginty’s team showed that these same touch neurons also innervate the colon, where their axons branch and curl around motor neurons in the gut, enabling us to sense bowel distention. “So you might say there are actually 50 or 60 different touch neuron types,” he said, if you count both genetic and physical variations. “We don’t know how many there are.”
Ginty will keep counting them. Today he’s asking the same fundamental questions he set out to answer more than a decade ago: Where do the various touch neurons go, what are their end structures, and how do they capture the richness of the physical realm? “We’ve gotten a pretty good handle on who’s who in the skin and what their response properties are,” Ginty said. But what about the heart, lungs, larynx, esophagus, stomach, intestines and kidneys? What are the neurons that make muscles ache and fatigue, or trigger migraines, or cause milk to flow in a mother’s breast when her baby suckles?
Ginty also wants to know how all these neurons connect to the brain to generate perceptions. How does pressure and vibration across millions of nerve endings become a hug? How do we feel wetness, slipperiness or elasticity? “Think about squeezing a balloon,” he said. “Presumably no one sensory neuron type is going to encode squeeziness.”
His work has transformed our understanding not only of individual touch sensors, but also of their connectivity. Until recently, the canonical view was that touch signals, like a telephone conversation, travel along fixed lines all the way to the somatosensory cortex, the part of the outer brain associated with sense information. “So any higher-order feature of the tactile world was seen as an emergent property of the cortex,” Ginty said. But his research and that of others has caused a paradigm shift. It’s now clear that a great deal of information carried by touch neurons converges in the spinal cord and brainstem before reaching the cognitive parts of the brain, suggesting that the touch signals are processed earlier in the neurobiological pathway than once believed.
If you ask Ginty what all this knowledge is good for, he’ll list the predictable applications: better pain drugs, improved treatments for sensory processing disorders such as autism, more lifelike prosthetics. But what really motivates him is something less tangible: awe. His work, he’ll tell you, has given him a deeper appreciation of this sense we so often take for granted — how nuanced and multidimensional it is, and how much it can still surprise him.
Not long ago, he said, he attended a performance of the Boston Symphony Orchestra. “I put my fingers on the chair, and I closed my eyes and just felt the music.”